In the previous entry I talked about the electrical signal or action potential that’s fired and acts as the initial communication in the nervous system. In this entry I’ll cover the network upon which that signal travels, as well as a few other related and interesting topics.
The Electrical Network
The network of our nervous system is not so different from the electrical system in our homes, it’s just a lot more complex. And gooey.
There is an overall structure:
- There is the central nervous system (CNS), which is comprised of our spinal cord and our brain, and is sort of like our fuse box.
- And then there are all the wires emanating from the CNS to our limbs and extremities. This is the peripheral nervous system (PNS) and is akin to the wiring that winds through the walls of a home. It starts at the point where nerves extend from the spinal cord and runs to the ends of our limbs. The sciatic nerve is probably the longest axon (nerve fiber), running from the lumbosacral plexus L4-S3 (you’ll understand what this means in few paragraphs believe it or not) to our big toe.
Also, the PNS is divided and categorized several times–first into the somatic (voluntary movement) and autonomic (involuntary movement) systems; and then the autonomic system is broken down into the sympathetic and parasympathetic systems. More on that later.
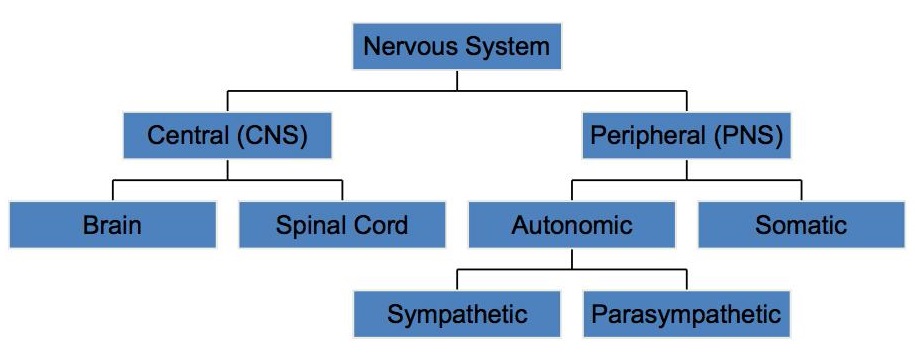
So, again thinking back to Part I of the Nervous System, the impetus to all activity in our bodies is a change in the action potential–a change in the electro-chemical charge of ions in, and surrounding, our cells and in this case, our neurons.
The action potential, or electrical charge, travels the length of an axon toward the brain. The signal makes connections along the way; first in the spinal cord, then in the thalamus, and lastly in the cerebral cortex.
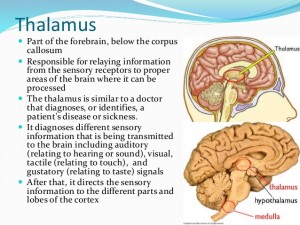
Different types of signals have different “tracts”, or paths on which they travel. For example, the spinothalamic tract conveys touch sensory data and crosses over–decussates–to the opposite side of the spinal cord before continuing on to the thalamus. So the message of a bump to the right leg will travel up the left side of the spinothalamic tract. It is an ascending tract, meaning that the signal is moving from the PNS to the CNS.
It’s important for medical professionals to know all the tracts and possible ways that information travels to and from the brain as it helps in narrowing down the specific location of a spinal cord injury. For example, some tracts communicate touch sensors, others pain, and others temperature, etc.
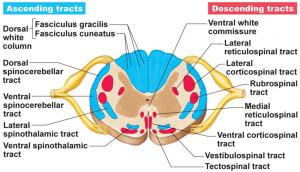
The central nervous system, or CNS, includes the brain and the spinal cord, which travels uninterrupted from the brain stem to the lumbar vertebrae. The spinal column is made up of 33 different bones called vertebrae, each with a hole in the center, through which the spinal cord travels. Of the 33 vertebrae, 7 are in the cervical region, 12 in the thoracic region, 5 in the lumbar region, 5 in the sacral region and 4 in the coccygeal region.
Most of us know that spinal injuries are very serious, but do we understand why? It’s not a broken vertebrae that serious although that shouldn’t be taken lightly–bones heal. Because nerve fibers in the central nervous system (brain and spinal cord) rarely regenerate, such damage is irreversible. If the spinal cord is severed, there is no neural communication getting past that point, which is why injuries higher up on the spine are more deadly; if the cord is injured above vertebrae C4, we lose neural communication and use of all limbs, but if the injury is below T11, we lose use our upper limbs only. The phrenic nerve, which extends from the area between vertebrae C3-C5, leads to our diaphragm and controls our breathing; obviously any damage above or to that nerve could be life threatening.
So far I’ve covered a lot on voluntary sensory communication–you get cold and put on a jacket, or your finger gets burned so you move it away from the heat–but a lot of our neural communication happens behind the scenes. The autonomic system that I mentioned earlier controls all involuntary neural communication. Again, it’s divided into the sympathetic and parasympathetic systems and they are almost opposite in their affect on the body.
Most of us are familiar with the sympathetic system as it’s commonly referred to as fight-or-flight; this system is activated involunarily, in emergency situations when we feel emotionally and/or physically threatened. It causes our endocrine system (more on that later) to release the hormone adrenaline (also known as epinephrine) which causes:
- accelerated heart rate,
- widened bronchial passages,
- decreased digestive function,
- constricted blood vessels,
- pupil dilation,
- goose bumps,
- perspiration (sweating), and
- raised blood pressure.
It basically mobilizes all our resources toward functions primarily tasked with quick thought and action.
Conversely, our parasympathetic system–also known as rest-and-digest– is working behind the scenes when we’re not stressed. It’s effects include:
- slower the heart rate,
- increased intestinal and gland activity,
- relaxed gastrointestinal tract
- sexual arousal
An important aspect of how an action potential travels on an axon is called saltatory conduction, which means that the action potential leaps across the axon as it travels. This greatly speeds up transmission of the signal and is integral to survival. This is possible due to the basic structure of the axon: it’s divided into sections by a type of fatty insulation called a myelin sheath. The small space in between each myelin sheath is the node of ranvier, and acts as the hopping point while the myelin insulates and accelerates the signal.
The movement of newborns is jerky because their myelin sheaths are not fully mature; as a result the stimulus or action potential travels along the axon in an irregular, uncoordinated way.
Many diseases are the result of demyelination, the most common being multiple sclerosis. In MS, the myelin sheaths in the CNS are damaged, which disrupts saltatory conduction, i.e., the ability of parts of the nervous system to communicate. The results are a range of signs and symptoms, including physical, mental, and sometimes psychiatric problems.
Parkinson’s is also a degenerative disorder of the nervous system, with progressive impairment or deterioration of neurons in an area of the brain known as the substantia nigra. This part of the brain plays an important role in reward and movement, which is why Parkinson’s sufferers not only have physical symptoms (shaking) but also commonly have problems with impulse control.
There is so much of the nervous system that I haven’t covered, but it’s time to move on. I welcome any questions, even on topics not included above–I could talk/write about this stuff all day. 🙂